From Wikipedia, the free
encyclopedia
"Bio-energy" redirects here. For the term
bio-energy in the context of non-mechanist
philosophy or alternative medicine, see
Vitalism.
Biofuel can be broadly defined as solid,
liquid, or gas
fuel derived from recently dead
biological material.This distinguishes it from
fossil fuels, which are
derived from long dead biological material.
Biofuel can be theoretically produced from any (biological)
carbon source, though the most common by far is
photosynthetic
plants. Many different plants and plant-derived
materials are used for biofuel manufacture. Biofuels
are used globally, most commonly to power
vehicles and cooking stoves. Biofuel industries
are expanding in Europe, Asia and the Americas.
Biofuels offer the possibility of producing
energy without a net increase of carbon into the
atmosphere because the plants used in to produce the
fuel have
removed CO2 from the atmosphere,
unlike fossil fuels which return carbon which was
stored beneath the surface for millions of years
into the air. Biofuel is therefore more nearly
carbon neutral and less likely increase
atmospheric concentrations of
greenhouse gases (though doubts have been raised
as to whether this benefit can be achieved in
practice,
see below). The use of biofuels also reduces
dependence on petroleum and enhances
energy security.
There are two common strategies of producing
biofuels. One is to grow crops high in either sugar
(sugar
cane,
sugar beet, and
sweet sorghum) or
starch (corn/maize),
and then use
yeast fermentation to produce ethyl alcohol (ethanol).
The second is to grow plants that contain high
amounts of vegetable oil, such as
oil palm,
soybean,
algae, or
jatropha. When these oils are heated, their
viscosity is reduced, and they can be burned
directly in a
diesel engine, or the oils can be chemically
processed to produce fuels such as
biodiesel. Wood and its byproducts can also be
converted into biofuels such as
woodgas,
methanol or
ethanol fuel. It is also possible to make
cellulosic ethanol from non-edible plant parts,
but this can be difficult to accomplish
economically.
Biofuels are discussed as having significant
roles in a variety of international issues,
including: mitigation of
carbon emissions levels and
oil prices, the "food
vs fuel" debate,
deforestation and
soil erosion, impact on
water resources, and energy balance and
efficiency.
History and
policy
Humans have used biomass fuels in the form of
solid biofuels for heating and cooking since the
discovery of fire. Following the discovery of
electricity, it became possible to use biofuels to
generate electrical power as well. However, the
discovery and use of
fossil fuels:
coal,
gas and
oil, have dramatically reduced the amount of
biomass fuel used in the developed world for
transport, heat and power. biofuels-p2.html National
Geographic, Green Dreams, Oct 2007]
However, when large
supplies of
crude oil were discovered in
Pennsylvania and
Texas,
petroleum based fuels became inexpensive, and
soon were widely used. Cars and trucks began using
fuels derived from
mineral oil/petroleum:
gasoline/petrol
or
diesel.
Nevertheless, before
World War II, and during the high demand wartime
period, biofuels were valued as a strategic
alternative to imported oil. Wartime Germany
experienced extreme oil shortages, and many energy
innovations resulted. This includes the powering of
some of its vehicles using a blend of gasoline with
alcohol fermented from potatoes, called Monopolin.[citation
needed] In Britain,
grain alcohol was blended with
petrol by the
Distillers Company Limited under the name
Discol, and marketed through
Esso's affiliate Cleveland.[citation
needed]
During the peacetime post-war period,
inexpensive oil from the
Middle East contributed in part to the lessened
economic and geopolitical interest in biofuels. Then
in 1973 and 1979, geopolitical conflict in the
Middle East caused
OPEC to cut exports, and non-OPEC nations
experienced a very large decrease in their oil
supply. This "energy
crisis" resulted in severe shortages, and a
sharp increase in the prices of high
demand oil-based products, notably
petrol/gasoline.
There was also increased interest from governments
and academics in energy issues and biofuels.
Throughout history, the fluctuations of
supply and demand,
energy policy,
military conflict, and the environmental
impacts, have all contributed to a highly complex
and volatile market for energy and fuel.
In the year 2000 and beyond, renewed interest
in biofuels has been seen. The drivers for biofuel
research and development include rising oil
prices, concerns over the potential
oil peak,
greenhouse gas emissions (causing
global warming and
climate change), rural development interests,
and instability in the Middle East.
Biomass
-
Biomass is material derived from recently
living
organisms. This includes plants, animals and
their by-products. For example, manure, garden waste
and crop residues are all sources of biomass. It is
a
renewable energy source based on the
carbon cycle, unlike other
natural resources such as
petroleum,
coal, and
nuclear fuels.
Animal waste is a persistent and unavoidable
pollutant produced primarily by the animals housed
in industrial sized farms. Researchers from
Washington University have figured out a way to turn
manure into
biomass. In April 2008 with the help of imaging
technology they noticed that vigorous mixing helps
microorganisms turn farm waste into alternative
energy, providing farmers with a simple way to treat
their waste and convert it into energy.
There are also
agricultural products specifically grown for
biofuel production include
corn,
switchgrass, and
soybeans, primarily in the United States;
rapeseed,
wheat and
sugar beet primarily in Europe;
sugar cane in Brazil;
palm oil and
miscanthus in South-East Asia;
sorghum and
cassava in China; and
jatropha in India.
Hemp has also been proven to work as a biofuel.
Biodegradable outputs from industry,
agriculture, forestry and households can be used for
biofuel production, either using
anaerobic digestion to produce
biogas, or using
second generation biofuels; examples include
straw, timber, manure, rice husks, sewage, and food
waste. The use of biomass fuels can therefore
contribute to waste management as well as fuel
security and help to prevent climate change, though
alone they are not a comprehensive solution to these
problems.
Bio energy from waste
Using waste biomass to produce energy can
reduce the use of fossil fuels, reduce greenhouse
gas emissions and reduce pollution and waste
management problems. A recent publication by the
European Union highlighted the potential for
waste-derived bioenergy to contribute to the
reduction of global warming. The report concluded
that 19 million tons of oil equivalent is available
from biomass by 2020, 46% from bio-wastes: municipal
solid waste (MSW), agricultural residues, farm waste
and other biodegradable waste streams.
Landfill sites generate gases as the waste
buried in them undergoes
anaerobic digestion. These gases are known
collectively as
landfill gas (LFG). This can be burned and is
considered a source of renewable energy, even though
landfill disposal are often non-sustainable.
Landfill gas can be burned either directly for heat
or to generate
electricity for public consumption. Landfill gas
contains approximately 50% methane, the same gas
that is found in
natural gas.
Biomass can come from waste plant material. If
landfill gas is not harvested, it escapes into the
atmosphere: this is not desirable because methane is
a greenhouse gas, with more
global warming potential than carbon dioxide.
Over a time span of 100 years, methane has a global
warming potential of 23 relative to CO2.
Therefore, during this time, one ton of methane
produces the same greenhouse gas (GHG) effect as 23
tons of CO2.[citation
needed] When methane burns the
formula is CH4 + 2O2 = CO2
+ 2H2O So by harvesting and burning
landfill gas, its global warming potential is
reduced a factor of 23, in addition to providing
energy for
heat and power.
Frank Keppler and Thomas Rockmann discovered
that living plants also produce methane CH4.
The amount of methane produced by living plants is
10 to 100 times greater than that produced by dead
plants (in an aerobic environment) but does not
increase global warming because of the
carbon cycle.
Anaerobic digestion can be used as a distinct
waste management strategy to reduce the amount of
waste sent to landfill and generate methane, or
biogas. Any form of biomass can be used in
anaerobic digestion and will break down to
produce
methane, which can be harvested and burned to
generate heat, power or to power certain automotive
vehicles.
A 3 MW landfill power plant would power 1,900
homes. It would eliminate 6,000 tons per year of
methane from getting into the environment..
It would eliminate 18,000 tons per year of CO2
from fossil fuel replacement.. This is
the same as removing 25,000 cars from the road,
or planting 36,000 acres (146 km²) of forest,
or not using 305,000 barrels (48,500 m³) of oil per
year.
Liquid fuels for
transportation
Most transportation fuels are liquids, because
vehicles usually require high
energy density, as occurs in
liquids and
solids. Vehicles usually need high
power density as can be provided most
inexpensively by an
internal combustion engine. These engines
require clean burning fuels, in order to keep the
engine clean and minimize
air pollution. The fuels that are easier to burn
cleanly are typically liquids and
gases. Thus liquids (and gases that can be
stored in liquid form) meet the requirements of
being both portable and clean burning. Also, liquids
and gases can be
pumped, which means handling is easily
mechanized, and thus less laborious.
Types of biofuels
First generation
biofuels
'First-generation biofuels' refer to biofuels
made from
sugar,
starch,
vegetable oil, or
animal fats using conventional technology.
The basic feedstocks for the production of
first generation biofuels are often seeds or grains
such as wheat, which yields starch that is fermented
into bioethanol, or sunflower seeds, which are
pressed to yield vegetable oil that can be used in
biodiesel. These feedstocks could also enter the
animal or human food chain, and as the global
population has risen their use in producing biofuels
has been criticised for diverting food away from the
human food chain, leading to food shortages and
price rises.
The most common first generation biofuels are
listed below.
Vegetable oil
-
Edible vegetable oil is generally not used as
fuel, but lower quality oil can be used for this
purpose. Used vegetable oil is increasingly being
processed into biodiesel, or (less frequently)
cleaned of water and particulates and used as a
fuel. To ensure that the fuel injectors atomize the
fuel in the correct pattern for efficient
combustion, vegetable oil fuel must be heated to
reduce its viscosity to that of diesel, either by
electric coils or heat exchangers. This is easier in
warm or temperate climates.
MAN B&W Diesel,
Wartsila and
Deutz AG offer engines that are compatible with
straight vegetable oil, without the need for after
market modifications. Vegetable oil can also be used
in many older diesel engines that do not use common
rail or unit injection electronic diesel injection
systems. Due to the design of the combustion
chambers in
indirect injection engines, these are the best
engines for use with vegetable oil. This system
allows the relatively larger oil molecules more time
to burn. However, a number of drivers have
successfully experimented with earlier pre- "pumpe
duse" VW TDI engines and other similar engines with
direct injection.
Biodiesel
-
Biodiesel is the most common biofuel in
Europe. It is produced from
oils or fats using
transesterification and is a liquid similar in
composition to fossil/mineral diesel. Its chemical
name is fatty acid methyl (or ethyl) ester (FAME).
Oils are mixed with sodium hydroxide and methanol
(or ethanol) and the chemical reaction produces
biodiesel (FAME) and
glycerol. One part glycerol is produced for
every 10 parts biodiesel. Feedstocks for biodiesel
include animal fats, vegetable oils,
soy,
rapeseed,
jatropha,
mahua,
mustard,
flax,
sunflower,
palm oil,
hemp,
field pennycress, and
algae. Pure biodiesel (B100) is by far the
lowest emission diesel fuel. Although
liquefied petroleum gas and hydrogen have
cleaner combustion, they are used to fuel much less
efficient petrol engines and are not as widely
available.
Biodiesel can be used in any
diesel engine when mixed with mineral diesel.
The majority of vehicle manufacturers limit their
recommendations to 15% biodiesel blended with
mineral diesel. In some countries manufacturers
cover their diesel engines under warranty for B100
use, although Volkswagen of Germany, for example,
asks drivers to make a telephone check with the VW
environmental services department before switching
to B100. B100 may become more viscous at lower
temperatures, depending on the feedstock used,
requiring vehicles to have fuel line heaters. In
most cases biodiesel is compatible with diesel
engines from 1994 onwards, which use 'Viton' (by
DuPont) synthetic rubber in their mechanical
injection systems. Electronically controlled 'common
rail' and 'pump duse' type systems from the late 90s
onwards (whose finely metered and atomized
multi-stage injection systems are very sensitive to
the viscosity of the fuel), may only use biodiesel
blended with conventional diesel fuel. Many of the
current generation of diesel engines are made so
that they can run on B100 without altering the
engine itself, although this can be dependent on the
fuel rail design.
Since biodiesel is an effective solvent and
cleans residues deposited by mineral diesel, engine
filters may need to be replaced more often as the
biofuel dissolves old deposits in the fuel tank and
pipes. It also effectively cleans the engine
combustion chamber of carbon deposits, helping to
maintain efficiency. In many European countries, a
5% biodiesel blend is widely used and is available
at thousands of gas stations. Biodiesel is also an
oxygenated fuel, meaning that it contains a
reduced amount of carbon and higher hydrogen and
oxygen content than fossil diesel. This improves the
combustion of fossil diesel and reduces the
particulate emissions from un-burnt carbon.
In the USA, more than 80% of commercial trucks
and city buses run on diesel. Therefore there is an
emerging U.S. biodiesel market, estimated to have
grown 200 percent from 2004 to 2005. "By the end of
2006 biodiesel production was estimated to increase
fourfold [from 2004] to more than 1 billion
gallons,".
Bioalcohols
-
Main article:
Alcohol fuel
Biologically produced
alcohols, most commonly
ethanol, and less commonly
propanol and
butanol, are produced by the action of
microorganisms and
enzymes through the fermentation of sugars or
starches (easiest), or cellulose (which is more
difficult).
Biobutanol (also called biogasoline) is often
claimed to provide a direct replacement for
gasoline, because it can be used directly in a
gasoline engine (in a similar way to biodiesel in
diesel engines).
Butanol is formed by
ABE fermentation (acetone, butanol, ethanol) and
experimental modifications of the process show
potentially high net energy gains with butanol as
the only liquid product. Butanol will produce more
energy and allegedly can be burned "straight" in
existing gasoline engines (without modification to
the engine or car), and is less corrosive and less
water soluble than ethanol, and could be distributed
via existing infrastructures.
DuPont and
BP are working together to help develop Butanol.
Ethanol fuel is the most common biofuel
worldwide, particularly
in Brazil.
Alcohol fuels are produced by fermentation of
sugars derived from
wheat,
corn,
sugar beets,
sugar cane,
molasses and any sugar or starch that
alcoholic beverages can be made from (like
potato and
fruit waste, etc.). The
ethanol production methods used are
enzyme digestion (to release sugars from stored
starches,
fermentation of the sugars,
distillation and
drying. The distillation process requires
significant energy input for heat (often
unsustainable
natural gas
fossil fuel, but cellulosic biomass such as
bagasse, the waste left after sugar cane is
pressed to extract its juice, can also be used more
sustainably).
Ethanol can be used in petrol engines as a
replacement for
gasoline; it can be mixed with gasoline to any
percentage. Most existing automobile petrol engines
can run on blends of up to 15% bioethanol with
petroleum/gasoline. Gasoline with ethanol added has
higher
octane, which means that your engine can
typically burn hotter and more efficiently. In high
altitude (thin air) locations, some states mandate a
mix of gasoline and ethanol as a winter
oxidizer to reduce atmospheric pollution
emissions.
Ethanol fuel has less
BTU energy content, which means it takes more
fuel (volume and mass) to go the same distance.
More-expensive premium fuels contain less, or no,
ethanol. In high-compression engines, less ethanol,
slower-burning premium fuel is required to avoid
harmful
pre-ignition (knocking). Very-expensive aviation
gasoline (Avgas) is 100 octane made from 100%
petroleum. The high price of zero-ethanol Avgas does
not include federal-and-state road-use taxes.
Ethanol is very
corrosive to fuel systems, rubber
hoses-and-gaskets,
aluminum, and
combustion chambers. It is therefore illegal to
use fuels containing alcohol in aircraft (although
at least one model of ethanol-powered aircraft has
been developed, the
Embraer EMB 202 Ipanema). Ethanol is
incompatible with marine fiberglass fuel tanks (it
makes them leak). For higher ethanol percentage
blends, and 100% ethanol vehicles, engine
modifications are required.
Corrosive ethanol cannot be transported in
petroleum pipelines, so more-expensive over-the-road
stainless-steel tank trucks increase the cost and
energy consumption required to deliver ethanol to
the customer at the pump.
In the current alcohol-from-corn production
model in the United States, considering the total
energy consumed by
farm equipment, cultivation, planting,
fertilizers,
pesticides,
herbicides, and
fungicides made from petroleum,
irrigation systems, harvesting, transport of
feedstock to processing plants,
fermentation,
distillation, drying, transport to fuel
terminals and retail pumps, and lower
ethanol fuel energy content, the net energy
content value added and delivered to consumers is
very small. And, the net benefit (all things
considered) does little to reduce un-sustainable
imported oil and fossil fuels required to produce
the ethanol.
Many car manufacturers are now producing
flexible-fuel vehicles (FFV's), which can safely
run on any combination of bioethanol and petrol, up
to 100% bioethanol. They dynamically sense exhaust
oxygen content, and adjust the engine's computer
systems, spark, and fuel injection accordingly. This
adds initial cost and ongoing increased vehicle
maintenance. Efficiency falls and pollution
emissions increase when FFV system maintenance is
needed (regardless of the 0%-to-100% ethanol mix
being used), but not performed (as with all
vehicles). FFV
internal combustion engines are becoming
increasingly complex, as are multiple-propulsion-system
FFV
hybrid vehicles, which impacts cost,
maintenance,
reliability, and useful lifetime
longevity
Alcohol mixes with both petroleum and with
water, so
ethanol fuels are often diluted after the drying
process by absorbing environmental moisture from the
atmosphere. Water in alcohol-mix fuels reduces
efficiency, makes engines harder to start, causes
intermittent operation (sputtering), and oxidizes
aluminum (carburetors)
and steel components (rust).
Even dry ethanol has roughly one-third lower
energy content per unit of volume compared to
gasoline, so larger / heavier fuel tanks are
required to travel the same distance, or more fuel
stops are required. With large current un-sustainable,
non-scalable
subsidies,
ethanol fuel still costs much more per unit of
distance traveled than current high gasoline prices
in the United States.
Methanol is currently produced from
natural gas, a non-renewable
fossil fuel. It can also be produced from
biomass as biomethanol. The
methanol economy is an interesting alternative
to the
hydrogen economy, compared to today's hydrogen
produced from
natural gas, but not
hydrogen production directly from water and
state-of-the-art clean
solar thermal energy processes.
BioGas
-
Biogas is produced by the process of
anaerobic digestion of
organic material by
anaerobes. It can be produced either from
biodegradable waste materials or by the use of
energy crops fed into
anaerobic digesters to supplement gas yields.
The solid byproduct,
digestate, can be used as a biofuel or a
fertilizer. In the UK, the National Coal Board
experimented with microorganisms that digested coal
in situ converting it directly to gases such as
methane.
Biogas contains
methane and can be recovered from industrial
anaerobic digesters and
mechanical biological treatment systems.
Landfill gas is a less clean form of biogas which is
produced in
landfills through naturally occurring anaerobic
digestion. If it escapes into the atmosphere it is a
potent
greenhouse gas.
Oils and gases can be produced from various
biological wastes:
-
Thermal depolymerization of waste can extract
methane and other oils similar to petroleum.
-
GreenFuel Technologies Corporation developed a
patented bioreactor system that uses nontoxic
photosynthetic algae to take in smokestacks flue
gases and produce biofuels such as biodiesel,
biogas and a dry fuel comparable to coal.
Solid biofuels
Examples include wood, grass cuttings,
domestic refuse, charcoal, and dried
manure.
Syngas
-
Main article:
Gasification
Syngas is produced by the combined processes of
pyrolysis, combustion, and
gasification. Biofuel is converted into
carbon monoxide and energy by pyrolysis. A
limited supply of oxygen is introduced to support
combustion. Gasification converts further organic
material to hydrogen and additional carbon monoxide.
The resulting gas mixture, syngas, is itself a
fuel. Using the syngas is more efficient than direct
combustion of the original biofuel; more of the
energy contained in the fuel is extracted.
Syngas may be burned directly in internal
combustion engines. The
wood gas generator is a wood-fueled gasification
reactor mounted on an internal combustion engine.
Syngas can be used to produce
methanol and
hydrogen, or converted via the
Fischer-Tropsch process to produce a synthetic
petroleum substitute. Gasification normally
relies on temperatures >700°C. Lower temperature
gasification is desirable when co-producing
biochar.
Second generation
biofuels
-
Supporters of biofuels claim that a more
viable solution is to increase political and
industrial support for, and rapidity of,
second-generation biofuel implementation from
non food crops, including
cellulosic biofuels.[18]
Second-generation biofuel production processes can
use a variety of
non food crops. These include waste biomass, the
stalks of wheat, corn, wood, and
special-energy-or-biomass crops (e.g.
Miscanthus). Second generation (2G) biofuels use
biomass to liquid technology, including
cellulosic biofuels from
non food crops.[19]
Many second generation biofuels are under
development such as
biohydrogen,
biomethanol,
DMF, Bio-DME,
Fischer-Tropsch diesel, biohydrogen diesel,
mixed alcohols and wood diesel.
Cellulosic ethanol production uses
non food crops or inedible waste products and
does not divert food away from the animal or human
food chain.
Lignocellulose is the "woody" structural
material of plants. This feedstock is abundant and
diverse, and in some cases (like citrus peels or
sawdust) it is a significant disposal problem.
Producing
ethanol from
cellulose is a difficult technical problem to
solve. In nature,
Ruminant livestock (like
cattle) eat grass and then use slow enzymatic
digestive processes to break it into
glucose (sugar). In
cellulosic ethanol laboratories, various
experimental processes are being developed to do
the same thing, and then the sugars released can be
fermented to make ethanol fuel.
Scientists also work on experimental
recombinant DNA
genetic engineering organisms that could
increase biofuel potential.
Third generation
biofuels
-
Algae fuel, also called oilgae or third
generation biofuel, is a biofuel from
algae. Algae are low-input/high-yield (30 times
more energy per acre than land)
feedstocks to produce biofuels and algae fuel
are
biodegradable:
- One advantage of many biofuels over most
other fuel types is that they are
biodegradable, and so relatively harmless to
the environment if spilled.
Second and third generation biofuels are also
called advanced biofuels.
On the other hand, an appearing fourth
generation is based in the conversion of
vegoil and
biodiesel into gasoline.
Fourth generation
biofuels
Craig Venter's company
Synthetic Genomics is genetically engineering
microorganisms to produce fuel directly from
carbon dioxide on an industrial scale.
Biofuels by country
Recognizing the importance of implementing
bioenergy, there are international organizations
such as IEA Bioenergy,
established in 1978 by the
OECD
International Energy Agency (IEA), with the aim
of improving cooperation and information exchange
between countries that have national programs in
bioenergy research, development and deployment. The
U.N. International Biofuels Forum is formed by
Brazil,
China,
India,
South Africa, the
United States and the
European Commission. The world leaders in
biofuel development and use are Brazil, United
States, France, Sweden and Germany.
- See
also:
Biodiesel around the world
Israel
IC Green Energy, a subsidiary of Israel Corp.,
aims by 2012 to process 4-5% of the global biofuel
market (~4 million tons). It is focused solely on
non-edible feedstock such as Jatropha, Castor,
cellulosic biomass and algae. In June 2008, Tel
Aviv-based Seambiotic and Seattle-based Inventure
Chemical announced a joint venture to use CO2
emissions-fed algae to make ethanol and biodiesel at
a biofuel plant in Israel.
China
In
China, the government is making E10 blends
mandatory in five provinces that account for 16% of
the nation's passenger cars. In Southeast Asia,
Thailand has mandated an ambitious 10% ethanol
mix in gasoline starting in 2007. For similar
reasons, the palm oil industry plans to supply an
increasing portion of national diesel fuel
requirements in
Malaysia and
Indonesia. In
Canada, the government aims for 45% of the
country’s gasoline consumption to contain 10%
ethanol by 2010.
India
-
In India, a bioethanol program calls for E5
blends throughout most of the country targeting to
raise this requirement to E10 and then E20.
Europe
The
European Union in its
biofuels directive (updated 2006) has set the
goal that for 2010 that each member state should
achieve at least 5.75% biofuel usage of all used
traffic fuel. By 2020 the figure should be 10%. As
of January 2008 these aims are being reconsidered in
light of certain environmental and social concerns
associated with biofuels such as rising food prices
and deforestation.
France
France is the second largest biofuel consumer
among the EU States in 2006. According to the
Ministry of Industry, France's consumption increased
by 62.7% to reach 682,000
toe (i.e. 1.6% of French fuel consumption).
Biodiesel represents the largest share of this (78%,
far ahead of bioethanol with 22%). The
unquestionable biodiesel leader in Europe is the
French company
Diester Industrie. In bioethanol, the French
agro-industrial group Téréos is increasing its
production capacities. Germany itself remained the
largest European biofuel consumer, with a
consumption estimate of 2.8 million tons of
biodiesel (equivalent to 2,408,000 toe), 0.71
million ton of vegetable oil (628.492 toe) and 0.48
million ton of bioethanol (307,200 toe).
Germany
The biggest biodiesel German company is
ADM Ölmühle Hamburg AG, which is a subsidiary of
the American group
Archer Daniels Midland Company. Among the other
large German producers,
MUW (Mitteldeutsche Umesterungswerke GmbH & Co
KG) and EOP Biodiesel AG. A major contender in terms
of bioethanol production is the German sugar
corporation,
Südzucker.
Spain
The Spanish group
Abengoa, via its American subsidiary Abengoa
Bioenergy, is the European leader in production of
bioethanol.
Sweden
-
The government in Sweden has together with BIL
Sweden, the national association for the automobile
industry, that are the automakers in Sweden started
the work to end oil dependency. One-fifth of cars in
Stockholm can run on alternative fuels, mostly
ethanol fuel. Also
Stockholm will introduce a fleet of Swedish-made
hybrid ethanol-electric buses. In 2005,
oil phase-out in Sweden by 2020 was announced.
United Kingdom
In the
United Kingdom the
Renewable Transport Fuel Obligation (RTFO)
(announced 2005) is the requirement that by 2010 5%
of all road vehicle fuel is renewable. In 2008 a
critical report by the
Royal Society stated that biofuels risk
failing to deliver significant reductions in
greenhouse gas emissions from transport and could
even be environmentally damaging unless the
Government puts the right policies in place.
Brazil
-
In Brazil, the government hopes to build on
the success of the Proálcool ethanol program by
expanding the production of biodiesel which must
contain 2% biodiesel by 2008, increasing to 5% by
2013.
Colombia
Colombia mandates the use of 10% ethanol in all
gasoline sold in cities with populations exceeding
500,000. In
Venezuela, the state oil company is supporting
the construction of 15 sugar cane distilleries over
the next five years, as the government introduces a
E10 (10% ethanol) blending mandate.
USA
-
In 2006, the
United States president
George W. Bush said in a
State of the Union speech that the US is
"addicted to oil" and should replace 75% of imported
oil by 2025 by alternative sources of energy
including biofuels.
Essentially all of the
ethanol fuel in the US is produced from
corn. Corn is a very energy intensive crop,
which requires one unit of fossil-fuel energy to
create just 0.9 to 1.3 energy units of ethanol.[36]
A senior member of the
House Energy and Commerce Committee Congressman
Fred Upton has introduced legislation to use at
least E10 fuel by 2012 in all cars in the USA.
The 2007-12-19 U.S.
Energy Independence and Security Act of 2007
requires American “fuel producers to use at least 36
billion gallons of biofuel in 2022. This is nearly a
fivefold increase over current levels.”[37]
This is causing a significant agricultural resource
shift away from food production to biofuels.
American food exports have decreased (increasing
grain prices worldwide), and US food imports have
increased significantly.
Most biofuels are not currently cost-effective
without significant subsidies. "America's ethanol
program is a product of government subsidies. There
are more than 200 different kinds, as well as a 54
cents-a-gallon tariff on imported ethanol. This
prices Brazilian ethanol out of an otherwise
competitive market. Brazil makes ethanol from
sugarcane rather than corn (maize), which has a
better
EROEI. Federal subsidies alone cost $7 billion a
year (equal to around $1.90 a gallon)."
General Motors is starting a project to
produce
E85 fuel from
cellulose ethanol for a projected cost of $1 a
gallon. This is optimistic however, because $1/gal
equates to $10/MBTU which is comparable to
woodchips at $7/MBTU or
cord wood at $6-$12/MBTU, and this does not
account for conversion losses and plant operating
and capital costs which are significant. The raw
materials can be as simple as corn stalks and scrap
petroleum-based vehicle tires, but used tires are an
expensive feedstock with other more-valuable uses.
GM has over 4 million E85 cars on the road now, and
by 2012 half of the production cars for the U.S.
will be capable of running on E85 fuel, however by
2012 the supply of ethanol will not even be close to
supplying this much E85. Coskata Inc. is building
two new plants for the ethanol fuel. Theoretically,
the process is claimed to be five times more energy
efficient than corn based ethanol, however it is
still in development and has not been proven to be
cost effective in a free market.
The greenhouse gas emissions are reduced by
86% for cellulose compared to corn’s 29% reduction.
Biofuels in developing
countries
Biofuel industries are becoming established in
many developing countries. Many developing countries
have extensive biomass resources that are becoming
more valuable as demand for biomass and biofuels
increases. The approaches to biofuel development in
different parts of the world varies. Countries such
as India and China are developing both bioethanol
and biodiesel programs. India is extending
plantations of
jatropha, an oil-producing tree that is used in
biodiesel production. The Indian sugar ethanol
program sets a target of 5% bioethanol incorporation
into transport fuel China is a major bioethanol
producer and aims to incorporate 15% bioethanol into
transport fuels by 2010. Costs of biofuel promotion
programs can be very high, though.
Amongst rural populations in developing
countries, biomass provides the majority of fuel for
heat and cooking. Wood, animal dung and crop
residues are commonly burned. Figures from the
International Energy Agency show that biomass energy
provides around 30% of the total primary energy
supply in developing countries; over 2 billion
people depend on biomass fuels as their primary
energy source.
The use of biomass fuels for cooking indoors
is a source of health problems and pollution. 1.3
million deaths were attributed to the use of biomass
fuels with inadequate ventilation by the
International Energy Agency in its World Energy
Outlook 2006. Proposed solutions include improved
stoves and alternative fuels. However, fuels are
easily damaged, and alternative fuels tend to be
expensive. Very low cost, fuel efficient, low
pollution biomass stove designs have existed since
1980 or earlier.[43]
Issues are a lack of education, distribution, excess
corruption, and very low levels of foreign aid.
People in
developing countries are often unable to afford
these solutions without assistance or financing such
as
microloans. Organizations such as
Intermediate Technology Development Group work
to make improved facilities for biofuel use and
better alternatives accessible to those who cannot
get them.
Current issues in
biofuel production and use
Biofuels are proposed as having such benefits
as: reduction of
greenhouse gas emissions, reduction of
fossil fuel use, increased national
energy security, increased
rural development and a sustainable fuel supply
for the future.
However, biofuel production is questioned from
a number of angles. The chairman of the
International Panel on Climate Change,
Rajendra Pachauri, notably observed in March
2008 that questions arise on the emissions
implications of that route, and that biofuel
production has clearly raised prices of corn, with
an overall implication for food security.
Biofuels are also seen as having limitations.
The feedstocks for biofuel production must be
replaced rapidly and biofuel production processes
must be designed and implemented so as to supply the
maximum amount of fuel at the cheapest cost, while
providing maximum environmental benefits. Broadly
speaking, first generation biofuel production
processes cannot supply us with more than a few
percent of our energy requirements sustainably. The
reasons for this are described below. Second
generation processes can supply us with more biofuel,
with better environmental gains. The major barrier
to the development of second generation biofuel
processes is their capital cost: establishing second
generation biodiesel plants has been estimated at
€500million.
Recently, an inflexion point about
advantages/disadvantages of biofuels seems to be
gaining momentum. The March 27, 2008
TIME magazine cover features the subject under
the title "The Clean Energy Myth":
Politicians and Big Business are pushing
biofuels like corn-based ethanol as alternatives
to oil. All they’re really doing is driving up
world food prices, helping to destroy the Amazon
jungle, and making global warming worse.
In the June, 2008 issue of the journal
Conservation Biology, scientists argue that because
such large amounts of energy are required to grow
corn and convert it to ethanol, the net energy gain
of the resulting fuel is modest. Using a crop such
as switchgrass, common forage for cattle, would
require much less energy to produce the fuel, and
using algae would require even less. Changing
direction to biofuels based on switchgrass or algae
would require significant policy changes, since the
technologies to produce such fuels are not fully
developed.
Oil price moderation
The
International Energy Agency's World Energy
Outlook 2006 concludes that rising oil demand,
if left unchecked, would accentuate the consuming
countries' vulnerability to a severe supply
disruption and resulting price shock. The report
suggested that biofuels may one day offer a viable
alternative, but also that "the implications of the
use of biofuels for global security as well as for
economic, environmental, and public health need to
be further evaluated".
Economists disagree on the extent that biofuel
production affects crude oil prices. According to
the Francisco Blanch, a commodity strategist for
Merrill Lynch, crude oil would be trading 15 per
cent higher and gasoline would be as much as 25 per
cent more expensive, if it were not for biofuels.
Gordon Quaiattini, president of the Canadian
Renewable Fuels Association, argued that a healthy
supply of alternative energy sources will help to
combat gasoline price spikes. However, the Federal
Reserve Bank of Dallas concluded that "Biofuels are
too limited in scale and currently too costly to
make much difference to crude oil pricing."
Rising food prices —
the "food vs. fuel" debate
-
Main article:
food vs fuel
This topic is internationally controversial.
There are those, such as the National Corn Growers
Association, who say biofuel is not the main cause.
Some say the problem is a result of government
actions to support biofuels. Others say it is just
due to oil price increases. The impact of food price
increases is greatest on poorer countries. Some have
called for a freeze on biofuels. Some have called
for more funding of second generation biofuels which
should not compete with food production so much.
In May 2008 Olivier de Schutter, the
United Nations food adviser, called for a halt
on biofuel investment. In an interview in
Le Monde he stated: "The ambitious goals for
biofuel production set by the United States and the
European Union are irresponsible. I am calling for a
freeze on all investment in this sector." 100
million people are currently at risk due to the food
price increases.
Carbon emissions
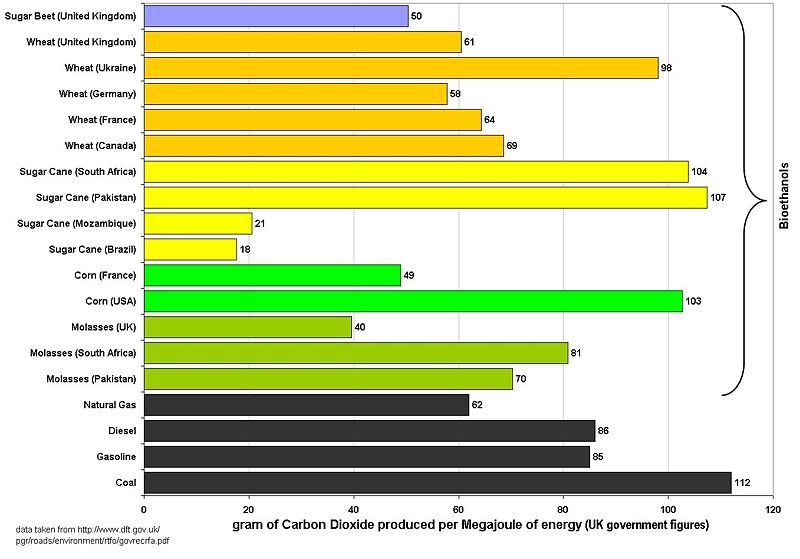
Graph of UK figures for the
carbon intensity of bioethanol and
fossil fuels. This graph assumes that all
bioethanols are burnt in their country of
origin and that prevously existing cropland is
used to grow the feedstock.
Biofuels and other forms of
renewable energy aim to be
carbon neutral or even carbon negative. Carbon
neutral means that the carbon released during the
use of the fuel, e.g. through burning to power
transport or generate electricity, is reabsorbed and
balanced by the carbon absorbed by new plant growth.
These plants are then harvested to make the next
batch of fuel.
Carbon neutral fuels lead to no net increases in
human contributions to atmospheric
carbon dioxide levels, reducing the human
contributions to
global warming. A carbon negative aim is
achieved when a portion of the biomass is used for
carbon sequestration. Calculating exactly how
much
greenhouse gas (GHG) is produced in burning
biofuels is a complex and inexact process, which
depends very much on the method by which the fuel is
produced and other assumptions made in the
calculation.
Carbon emissions have been increasing ever
since the industrial revolution. Prior to the
industrial revolution, our atmosphere contained
about 280 parts per million of carbon dioxide. After
burning coal, gas, and oil to power our lives, the
concentration had risen to 315 parts per million.
Today, it is at the 380 level and still increasing
by approximately two parts per million annually.
During this time frame, the global average
temperature has risen by more than 1°F since carbon
dioxide traps heat near the Earth’s surface.
Scientists believe that if the level goes beyond 450
parts per million, the temperature jump will be so
great that we will be faced with an enormous rise in
sea level due to the melting of Greenland and West
Antarctic ice sheets.
The carbon emissions (Carbon
footprint) produced by biofuels are calculated
using a technique called
Life Cycle Analysis (LCA). This uses a "cradle
to grave" or "well to wheels" approach to calculate
the total amount of carbon dioxide and other
greenhouse gases emitted during biofuel production,
from putting seed in the ground to using the fuel in
cars and trucks. Many different LCAs have been done
for different biofuels, with widely differing
results. The majority of LCA studies show that
biofuels provide significant greenhouse gas
emissions savings when compared to fossil fuels such
as petroleum and diesel.[citation
needed] Therefore, using
biofuels to replace a proportion of the fossil fuels
that are burned for transportation can reduce
overall greenhouse gas emissions. The
well-to-wheel analysis for biofuels has shown
that first generation biofuels can save up to 60%
carbon emission and second generation biofuels can
save up to 80% as opposed to using fossil fuels.
However these studies do not take into account
emissions from nitrogen fixation, deforestation,
land use, or any indirect emissions.
In October 2007, a study was published by
scientists from Britain, U.S., Germany and Austria,
including Professor
Paul Crutzen, who won a Nobel Prize for his work
on ozone. They reported that the burning of biofuels
derived from
rapeseed and
corn (maize) can contribute as much or more to
global warming by
nitrous oxide emissions than cooling by fossil
fuel savings. Nitrous oxide is both a potent
greenhouse gas and a destroyer of atmospheric ozone.
But they also reported that crops with lower
requirements for
nitrogen
fertilizers, such as grasses and woody
coppicing will result in a net absorption of
greenhouse gases.
In February 2008, two articles were published
in
Science which investigated the GHG emissions
effects of the large amount of natural land that is
being converted to cropland globally to support
biofuels development. The first of these studies,
conducted at the
University of Minnesota, found that:
...converting rainforests, peatlands,
savannas, or grasslands to produce food-based
biofuels in Brazil, Southeast Asia, and the United
States creates a ‘biofuel carbon debt’ by
releasing 17 to 420 times more CO2 than
the annual greenhouse gas (GHG) reductions these
biofuels provide by displacing fossil fuels.
This study not only takes into account removal
of the original vegetation (as timber or by burning)
but also the biomass present in the soil, for
example roots, which is released on continued
plowing. It also pointed out that:
...biofuels made from waste biomass or from
biomass grown on degraded and abandoned
agricultural lands planted with perennials incur
little or no carbon debt and can offer immediate
and sustained GHG advantages.
The second study, conducted at
Princeton University, used a worldwide
agricultural model to show that:
...corn-based ethanol, instead of producing
a 20% savings, nearly doubles greenhouse emissions
over 30 years and increases greenhouse gases for
167 years.
Both of the Science studies highlight the need
for
sustainable biofuels, using feedstocks that
minimize competition for prime croplands. These
include farm, forest and municipal waste streams;
energy crops grown on marginal lands, and algaes.
These second generation biofuels feedstocks "are
expected to dramatically reduce GHGs compared to
first generation biofuels such as corn ethanol". In
short, biofuels done unsustainably could make the
climate problem worse, while biofuels done
sustainably could play a leading role in solving the
carbon challenge.
Sustainable biofuel
production
-
Responsible policies and economic instruments
would help to ensure that biofuel commercialization,
including the development of new
cellulosic technologies, is
sustainable. Sustainable biofuel production
practices would not hamper food and fibre
production, nor cause water or environmental
problems, and would actually enhance soil
fertitlity. Responsible commercialization of
biofuels represents an opportunity to enhance
sustainable economic prospects in Africa, Latin
America and impoverished Asia.
Soil erosion,
deforestation, and biodiversity
It is important to note that
carbon compounds in waste
biomass that is left on the ground are consumed
by other
microorganisms. They break down
biomass in the soil to produce valuable
nutrients that are necessary for future crops.
On a larger scale, plant biomass waste provides
small wildlife
habitat, which in turn ripples up through the
food chain. The widespread human use of biomass
(which would normally
compost the field) would threaten these
organisms and natural habitats. When
cellulosic ethanol is produced from feedstock
like
switchgrass and
saw grass, the nutrients that were required to
grow the
lignocellulose are removed and cannot be
processed by microorganisms to replenish the soil
nutrients. The soil is then of poorer quality. Loss
of ground cover root structures accelerates
unsustainable
soil erosion.
Significant areas of native
Amazon rainforest have been cleared by
slash and burn techniques to make room for
sugar cane production, which is used in large
part for
ethanol fuel in Brazil, and growing
ethanol exports. Large-scale
deforestation of mature trees (which help remove
CO2 through
photosynthesis — much better than does
sugar cane or most other biofuel feedstock crops
do) contributes to un-sustainable
global warming atmospheric
greenhouse gas levels, loss of
habitat, and a reduction of valuable
biodiversity. Demand for biofuel has led to
clearing land for
Palm Oil plantations.
A portion of the biomass should be retained
onsite to support the soil resource. Normally this
will be in the form of raw biomass, but processed
biomass is also an option. If the exported biomass
is used to produce
syngas, the process can be used to co-produce
biochar, a low-temperature charcoal used as a
soil amendment to increase soil organic matter to a
degree not practical with less recalcitrant forms of
organic carbon. For co-production of biochar to be
widely adopted, the soil amendment and carbon
sequestration value of co-produced charcoal must
exceed its net value as a source of energy.
Impact on water
resources
Increased use of biofuels puts increasing
pressure on water resources in at least two ways:
water use for the irrigation of crops used as
feedstocks for biodiesel production; and water use
in the production of biofuels in refineries, mostly
for boiling and cooling.
In many parts of the world supplemental or
full irrigation is needed to grow feedstocks. For
example, if in the production of corn (maize) half
the water needs of crops are met through irrigation
and the other half through rainfall, about 860
liters of water are needed to produce one liter of
ethanol.
In the United States, the number of ethanol
factories has almost tripled from 50 in 2000 to
about 140 in 2008. A further 60 or so are under
construction, and many more are planned. Projects
are being challenged by residents at courts in
Missouri (where water is drawn from the
Ozark Aquifer), Iowa, Nebraska, Kansas (all of
which draw water from the non-renewable
Ogallala Aquifer), central Illinois (where water
is drawn from the
Mahomet Aquifer) and Minnesota.
Aldehydes
Formaldehyde,
Acetaldehyde and other
Aldehydes are produced when alcohols are
oxidized. When only a 10% mixture of ethanol is
added to gasoline (as is common in American
E10
gasohol and elsewhere), aldehyde emissions
increase 40%. Some study results are conflicting on
this fact however, and lowering the sulfur content
of biofuel mixes lowers the acetaldehyde levels.
Burning biodiesel also emits aldehydes and other
potentially hazardous aromatic compounds which are
not regulated in emissions laws.
Many aldehydes are toxic to living cells.
Formaldehyde irreversibly cross-links
protein
amino acids, which produces the hard flesh of
embalmed bodies. At high concentrations in an
enclosed space, formaldehyde can be a significant
respiratory irritant causing nose bleeds,
respiratory distress, lung disease, and persistent
headaches. Acetaldehyde, which is produced in the
body by alcohol drinkers and found in the mouths of
smokers and those with poor oral hygene, is
carcinogenic and
mutagenic.
The
European Union has banned products that contain
Formaldehyde, due to its documented
carcinogenic characteristics. The U.S.
Environmental Protection Agency has labeled
Formaldehyde as a probable cause of cancer in
humans.
Brazil burns significant amounts of ethanol
biofuel. Gas
chromatograph studies were performed of ambient
air in São Paulo Brazil, and compared to Osaka
Japan, which does not burn ethanol fuel. Atmospheric
Formaldehyde was 160% higher in Brazil, and
Acetaldehyde was 260% higher.
Social and Water
impact in Indonesia
In some locations such as Indonesia
deforestation for Palm Oil plantations is leading to
displacement of Indigenous peoples. Also, extensive
use of pesticide for biofuel crops is reducing clean
water supplies.
Environmental
organizations stance
Some mainstream environmental groups support
biofuels as a significant step toward slowing or
stopping global climate change.[citation
needed] However, biofuel
production can threaten the environment if it is not
done sustainably. This finding has been backed by
reports of the
UN, the
IPCC, and some other smaller environmental and
social groups as the
EEB and
the Bank Sarasin, which generally remain negative
about biofuels.
As a result, governmentaland environmental
organisations are turning against biofuels made at a
non-sustainable way (hereby preferring certain oil
sources as
jatropha and
lignocellulose over
palm oil) and are asking for global support for
this. Also, besides supporting these more
sustainable biofuels, environmental organisations
are redirecting to new technologies that do not use
internal combustion engines such as
hydrogen and compressed air.
The "Roundtable on Sustainable Biofuels" is an
international initiative which brings together
farmers, companies, governments, non-governmental
organizations, and scientists who are interested in
the sustainability of biofuels production and
distribution. During 2008, the Roundtable is
developing a series of principles and criteria for
sustainable biofuels production through
meetings, teleconferences, and online discussions.
The increased manufacture of biofuels will
require increasing land areas to be used for
agriculture. Second and third generation biofuel
processes can ease the pressure on land, because
they can use waste biomass, and existing (untapped)
sources of biomass such as crop residues and
potentially even marine algae.
In some regions of the world, a combination of
increasing demand for food, and increasing demand
for biofuel, is causing deforestation and threats to
biodiversity. The best reported example of this is
the expansion of oil palm plantations in Malaysia
and Indonesia, where rainforest is being destroyed
to establish new oil palm plantations. It is an
important fact that 90% of the palm oil produced in
Malaysia is used by the food industry; therefore
biofuels cannot be held solely responsible for this
deforestation. There is a pressing need for
sustainable palm oil production for the food and
fuel industries; palm oil is used in a wide variety
of food products. The Roundtable on Sustainable
Biofuels is working to define criteria,
standards and processes to promote sustainably
produced biofuels.
Palm oil is also used in the manufacture
of detergents, and in electricity and heat
generation both in Asia and around the world (the UK
burns palm oil in coal-fired power stations to
generate electricity).
Significant area is likely to be dedicated to
sugar cane in future years as demand for ethanol
increases worldwide. The expansion of sugar cane
plantations will place pressure on
environmentally-sensitive native ecosystems
including rainforest in South America. In forest
ecosystems, these effects themselves will undermine
the climate benefits of alternative fuels, in
addition to representing a major threat to global
biodiversity.
Although biofuels are generally considered to
improve net carbon output, biodiesel and other fuels
do produce local air pollution, including
nitrogen oxides, the principal cause of
smog.
Potential for poverty
reduction
Researchers at the
Overseas Development Institute have argued that
biofuels could help to reduce poverty in the
developing world, through increased
employment, wider
economic growth multipliers and energy price
effects. However, this potential is described as
'fragile', and is reduced where feedstock production
tends to be large scale, or causes pressure on
limited agricultural resources: capital investment,
land, water, and the net cost of food for the poor.
With regards to the potential for poverty
reduction or exacerbation, biofuels rely on many of
the same policy, regulatory or investment
shortcomings that impede
agriculture as a route to
poverty reduction. Since many of these
shortcomings require policy improvements at a
country level rather than a global one, they argue
for a country-by-country analysis of the potential
poverty impacts of biofuels. This would consider,
among other things, land administration systems,
market coordination and prioritising investment in
biodiesel, as this 'generates more labour, has
lower transportation costs and uses simpler
technology'.
Biofuel prices
Retail, at the pump prices, including U.S.
subsidies, Federal and state
motor taxes, B2/B5 prices for low-level
Biodiesel (B2-B5) are lower than
petroleum diesel by about 12 cents, and B20
blends are the same per unit of volume as
petrodiesel.
Due to the 1/3 lower energy content of
ethanol fuel, even the heavily-subsidized net
cost to drive a specific distance in
flexible-fuel vehicles is higher than current
gasoline prices.
Energy efficiency and
energy balance of biofuels
Production of biofuels from raw materials
requires
energy (for farming, transport and conversion to
final product, and the production / application of
fertilizers,
pesticides,
herbicides, and
fungicides), and has environmental consequences.
The
energy balance of a biofuel is determined by the
amount of energy put into the manufacture of fuel
compared to the amount of energy released when it is
burned in a vehicle. This varies by feedstock and
according to the assumptions used. Biodiesel made
from sunflowers may produce only 0.46 times the
input rate of fuel energy. Biodiesel made from
soybeans may produce 3.2 times the input rate of
fossil fuels. This compares to 0.805 for gasoline
and 0.843 for diesel made from petroleum. Biofuels
may require higher energy input per unit of
BTU energy content produced than
fossil fuels: petroleum can be pumped out of the
ground and processed more efficiently than biofuels
can be grown and processed. However, this is not
necessarily a reason to use oil instead of biofuels,
nor does it have an impact on the environmental
benefits provided by a given biofuel.
Studies have been done that calculate energy
balances for biofuel production. Some of these show
large differences depending on the biomass feedstock
used and location.
To explain one specific example, a
June 17,
2006 editorial in the Wall. St. Journal stated,
"The most widely cited research on this subject
comes from Cornell's David Pimental and Berkeley's
Ted Patzek. They've found that it takes more than a
gallon of fossil fuel to make one gallon of ethanol
— 29% more. That's because it takes enormous amounts
of fossil-fuel energy to grow corn (using fertilizer
and irrigation), to transport the crops and then to
turn that corn into ethanol."]
Life cycle assessments of biofuel production
show that under certain circumstances, biofuels
produce only limited savings in energy and
greenhouse gas emissions. Fertiliser inputs and
transportation of biomass across large distances can
reduce the GHG savings achieved. The location of
biofuel processing plants can be planned to minimize
the need for transport, and agricultural regimes can
be developed to limit the amount of fertiliser used
for biomass production. A European study on the
greenhouse gas emissions found that
well-to-wheel (WTW) CO2 emissions of
biodiesel from seed crops such as
rapeseed could be almost as high as fossil
diesel. It showed a similar result for bio-ethanol
from starch crops, which could have almost as many
WTW CO2 emissions as fossil petrol. This
study showed that second generation biofuels have
far lower WTW CO2 emissions.
Other independent LCA studies show that
biofuels save around 50% of the CO2
emissions of the equivalent fossil fuels. This can
be increased to 80-90% GHG emissions savings if
second generation processes or reduced fertiliser
growing regimes are used. Further GHG savings can be
achieved by using by-products to provide heat, such
as using
bagasse to power ethanol production from
sugarcane.
Collocation of
synergistic processing plants can enhance
efficiency. One example is to use the exhaust heat
from an industrial process for ethanol production,
which can then
recycle cooler processing water, instead of
evaporating hot water that warms the atmosphere.
Biofuels and solar
energy efficiency
Biofuels from plant materials convert energy
that was originally captured from
solar energy via
photosynthesis. A comparison of conversion
efficiency from solar to usable energy (taking into
account the whole energy budgets) shows that
photovoltaics are 100 times more efficient than
corn ethanol[104]
and 10 times more efficient than the best biofuel
Centralised vs.
decentralised production
There is debate around the best model for
production.
One side sees centralised vegetable oil fuel
production offering
- efficiency
- greater potential for fuel standardisation
- ease of administrating taxes
- possibility for rapid expansion
The other side of the argument points to
- increased fuel security
- rural job creation
- less of a 'monopolistic'
or 'oligopolistic'
market due to the increased number of producers
- benefits to local economy as a greater part
of any profits stay in the local economy
- decreased transportation and
greenhouse gases of feedstock and end product
- consumers close to and able to observe the
effects of production
The majority of established biofuel markets
have followed the centralised model with a few small
or micro producers holding a minor segment of the
market. A noticeable exception to this has been the
pure plant oil (PPO) market in Germany which grew
exponentially until the beginning of 2008 when
increasing feedstock prices and the introduction of
fuel duty combined to stifle the market. Fuel was
produced in hundreds of small oil mills distributed
throughout Germany often run as part of farm
businesses.
Initially fuel quality could be variable but
as the market matured new technologies were
developed that made significantly improvements. As
the technologies surrounding this fuel improved
usage and production rapidly increased with rapeseed
oil PPO forming a significant segment of
transportation biofuels consumed in 2007.
See also